A New Take on the Oldest Physics: What Actually Happened Right After the Big Bang?
April 7, 2025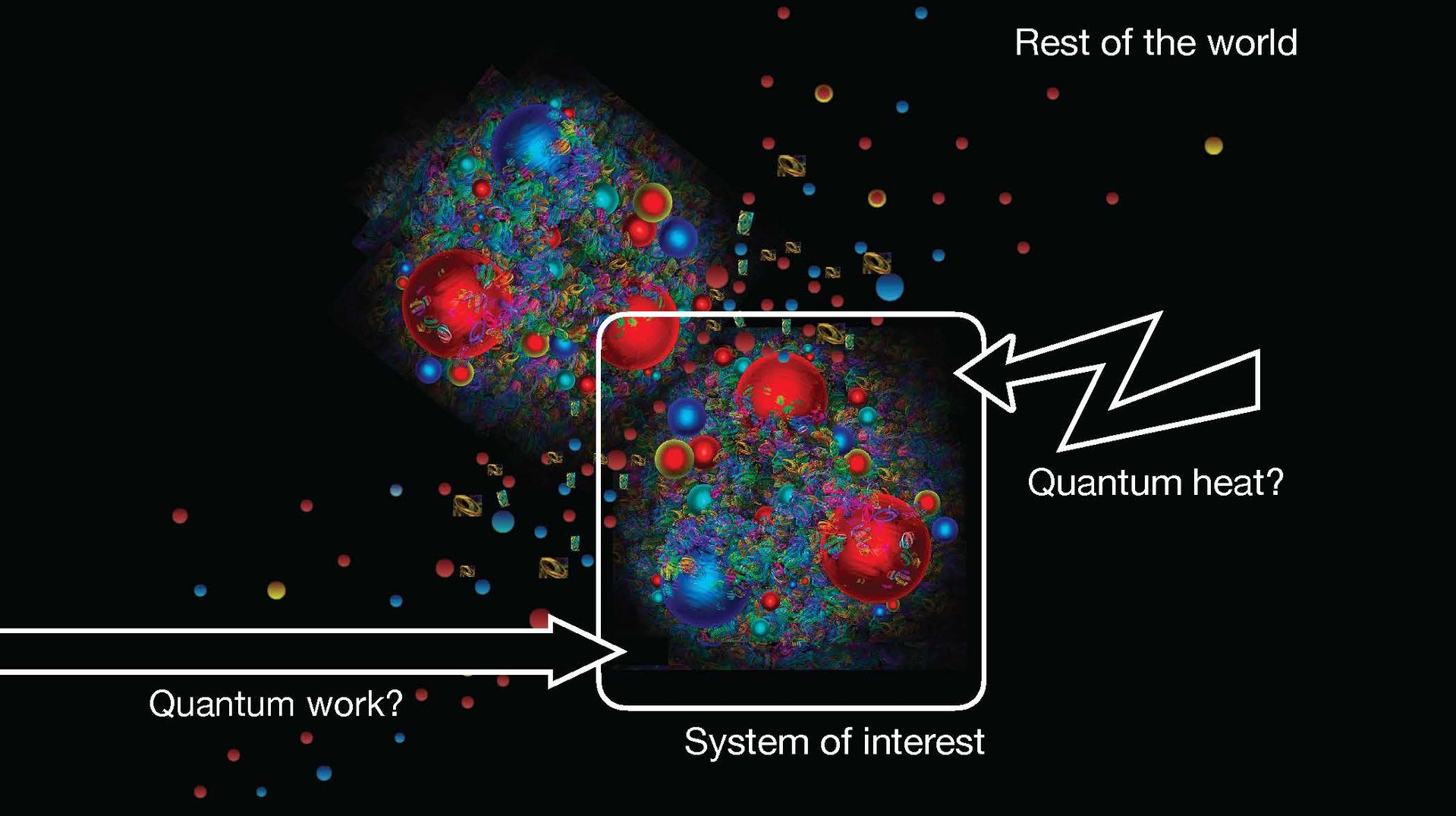
High-energy particle collisions, such as proton-proton collisions at the Large Hadron Collider, mimic the conditions in the early universe. These processes are governed by the quantum theories of strong, weak, and electromagnetic forces. A team of researchers at the Institute for Robust Quantum Simulation have shown how to define quantum-thermodynamic properties of systems described by such theories. They have further proposed ways to measure such quantities in quantum simulations. (Credit: Z. Davoudi/UMD with elements adopted from D. Dominguez/CERN).
Some of the biggest questions in physics—what happened in the first moments after the Big Bang? How do particles behave in the extreme conditions of high-energy collisions inside hadron colliders?—are too complex to fully calculate with today’s computers. A new collaboration at the Institute for Robust Quantum Simulation (RQS) has brought together seemingly unrelated fields to move toward tackling these questions in a new way.
By blending three disparate fields of physics—the study of elementary particles and their interactions, the understanding of temperature and heat in quantum mechanics, and quantum simulation—the collaborative team created a novel approach to understanding and modeling the physics of the early universe and high-energy collisions in particle accelerators, described recently in a publication in the journal Physical Review Letters.
“I was extremely happy about this connection,” says RQS senior investigator Nicole Yunger Halpern, a physicist at the National Institute of Standards and Technology. “I love quantum thermodynamics, and want more people to love it. So I want it to be applied far outside of its own neighborhood.”
The collaboration started from a series of conversations between Yunger Halpern and Zohreh Davoudi, the RQS associate director for education and a nuclear physicist at the University of Maryland.
Davoudi was coming from the world of nuclear and particle physics, trying to understand what exactly goes on inside of particle accelerators and, more ambitiously, what was happening in the first fractions of a second after the big bang. These settings are well described by the Standard Model of particle physics—physicists’ current best theory that unifies three of the four fundamental forces of nature: electromagnetism, the strong force that binds nuclei together, and the weak force responsible for radioactive decay. Gravity is the only oddball force that doesn’t fit into this framework.
The Early Universe Conundrum
The Standard Model dictates the equations that govern things like the early universe and high-energy particle collisions. However, actually using Standard Model equations to calculate what happens in realistic situations is another matter altogether. Doing calculations of how matter evolves in these extreme conditions takes all the computing power of today’s most powerful supercomputers, and even then, they are only able to tackle specific approximate solutions.
“It's a very hard problem, not only because these are very complex systems.” Davoudi says. “But also because it's non-equilibrium physics. Doing non-equilibrium, time-dependent quantum physics is just hard.”
Davoudi explained a particular complexity to Yunger Halpern when they met several years ago. In the Standard Model, every object in space is subject to rules known as Gauss’s laws that intertwine the fates of disparate charged particles via force fields.
These simple rules have complicated consequences: it means that to know the electric field at any particular point, one theoretically needs to know all the charges everywhere in space. Under these conditions, it’s tricky to isolate a region of space, restrict your calculations to only that region, and still get the right answer.
“These local constraints make it so that everything is interdependent on everything else,” Yunger Halpern says.
The Thermodynamic Solution
Upon hearing Davoudi’s description, Yunger Halpern realized there is a strong parallel between this problem and one she was interested in: so-called ‘strong-coupling thermodynamics.’
Thermodynamics is the study of heat, temperature, and work. One of the most basic assumptions of thermodynamics is that you can split the world into two pieces: the system, which is the little corner of the world you want to study, and the environment, which is everything else. Usually, physicists are able to use this separation to understand what’s going on in the system in detail and model the environment as a simple unified entity, say a background temperature, that has very limited effects on the system.
This separation makes a lot of sense in most cases. If you want to study what’s happening in your room, you can model the rest of the world just by the temperature outside. The air molecules flying around your room will only interact with the outside temperature when they hit the window, which is relatively straightforward to model.
However, there are more niche situations where this separation between system and environment cannot be made so cleanly. If, hypothetically, a tiny room had many huge windows, all of them open, plus the air particles impacted other air particles that were far away, strong coupling thermodynamics would kick in. This situation is contrived, but it does occur within the Standard Model, amongst elementary particles.
The field of strong-coupling thermodynamics provides a set of mathematical tools that allow scientists to effectively rejigger their model of the internal physics of the system of interest such that the environment, and its non-trivial interaction with the system, is taken into account.
Davoudi and Yunger Halpern decided to team up to see if the toolkit of strong coupling thermodynamics would also be useful for separating out the system and environment even when they are tightly interdependent. But this involved first taking the whole framework into the quantum realm, that realm of microscopic physics where the Standard Model resides. In this realm, particles can act like waves, changing the underlying mathematics.
The team quickly realized that the strong coupling thermodynamics toolkit wasn’t quite well developed enough to be used for their purposes. “People were using classical definitions of work and heat, and there were a number of them that were thought to be equivalent,” Davoudi says. “But they didn't realize that if you go to the quantum setting, those definitions are not equivalent. In fact, one set of them didn’t even satisfy the laws of thermodynamics in the quantum realm.”
As a first step, the team enlisted the help of another RQS senior investigator, Christopher Jarzynski, and graduate student Greeshma Oruganti, to extend the basic definitions of what work and heat is into the strong-coupling quantum realm in a way that made sense. This work resulted in another publication, an off-shoot of the original collaboration.
The Quantum Connection
Definitions in hand, the team tackled applying them to theories resembling those underlying physics of the early universe. This task largely fell to a former postdoc in Davoudi’s group named Niklas Mueller. Mueller is now an assistant professor at the University of New Mexico, focused on quantum information and simulation.
Mueller looked at the tools Davoudi and Yunger Halpern and their collaborators had in hand, and noticed that one particular quantity was extremely similar to a commonly used quantity in quantum information theory. The effective energy of the system with the strongly-coupled environment cleverly folded in was directly related to a quantity that measures entanglement—that quantum connection that makes remote particles act in sync.
“We were very excited by finding this relation,” Davoudi says, “because it means that if we want to measure things like work and heat in quantum simulation experiments, or using quantum computers, in principle, we can.”
The simple synergy
To test their newfound theoretical tools, the team came up with a toy problem. The toy problem maintained the basic features that make calculating predictions about the early universe difficult: Intertwining charge-field laws make it tricky to separate the system from the environment.
First, they confined the problem to one dimension—along a single line instead of in 3-dimensional space. Then, they narrowed the problem further to just six spins of one type (representing matter objects) on a ring, with six spins of another type (representing the force objects) sitting in between them. What happens to each spin depends inextricably on all the other dots making it hard to separate a ‘system’ from an ‘environment’.
Graduate student Connor Powers, aided by the rest of the team, performed computer simulations of this toy model. He extracted the thermodynamic quantities from the solution. They found that using their definitions of work and heat, they were able to see a phase transition, much like the change from liquid to gas.
Now, the team is planning to test out their ideas in a real quantum simulation experiment. “We have many of the pieces of the puzzle,” Davoudi says, “and I think it's just a matter of going to the experimentalists and asking our friends if they're interested.”
Davoudi acknowledges there is a long road ahead before these ideas can be used to shed light on the early universe. However, this is a novel path to tackle these problems. Davoudi believes this is a direct result of the collaborative environment at RQS. The most exciting part of the work was “just the idea of bridging two different fields of physics in a way we were learning each other's language and creating a common language together,” says Oruganti. Former graduate student Connor Powers, who also participated in the effort, agreed. “It was very cool to be a part of this bridge forming between these disciplines,” he says. “It was a really great opportunity.”
Experts
People
Zohreh Davoudi
Associate Director for Education